Introduction
Computed tomography (CT) is a fast, low-cost, and widely available diagnostic imaging modality. There is increasing concern about radiation-related side effects, especially in children [1]. Repeated CT examinations cause cumulative exposure to ionizing radiation in children, rendering them vulnerable to malignancies later in life, which are actually preventable. The children also have relatively fast-growing cells, and therefore they are inherently more likely to develop malignancies related to radiation exposure [1].
No other radiological investigation has greater radiation risk than that associated with CT exams. Due to the concerns associated with exposure to ionizing radiation, strategies are employed to minimize the radiation dose received during CT examinations, but not at the cost of compromising the quality of imaging and diagnostic results. The ALARA principle should be applied rigorously in children [1-4]. It is well documented that with correct justification, optimisation, and dose limitation, radiation risks can largely be reduced, if not totally eliminated. Certainly, in children, much more effort is required to guarantee judicious use of CT scans. In recent times, different software and methods for dose reduction have been developed by CT manufacturers [5-7].
X-ray tube current modulation and kVp optimization have been tried, with some success, for the reduction of the radiation dose, but these have their limitations and may compromise the image quality (because noise is increased) as well as the diagnostic value. Furthermore, noise reduction mathematical algorithms can be applied, which further reduces the radiation dose and simultaneously maintains the image quality by reducing the noise [5-7].
Filtered back projection (FBP) was the earliest of the noise reduction mathematical algorithms used in the image reconstruction. The iterative reconstruction (IR) technique has been a recent advancement in this approach. Due to the simple mathematical computation requirement, analytical reconstruction algorithms like FBP have been used to produce CT images, instead of IR algorithms [8,9]. Over the past few years, several IR algorithms have emerged in clinical CT applications. In recent years, model-based iterative reconstruction (MBIR), which is also known as a pure IR algorithm, has been shown to improve the quality of the images significantly, reducing noise and artifacts [9,10]. It also improves the spatial resolution. Some studies have been published to evaluate the effectiveness of MBIR and have shown promising results [11-14]. CT head is one of the most common CT examinations performed in children. The present prospective study was conducted to evaluate the potential of MBIR technique on dose reduction and image quality in children undergoing CT head examinations.
Material and methods
This was a prospective research study conducted over a period of 12 months. Our study was approved by the ethics committee of our institute. Patient confidentiality was maintained in accordance with Health Insurance Portability and Accountability Act guidelines (HIPAA). Informed written consent was taken from the parents/guardians of all the children. Assent was also obtained from the children aged 7 years and above prior to enrolment.
Eighty-eight consecutive children referred for contrast enhanced CT of the head were enrolled in this study.
Inclusion criteria:
age: 5-16 years,
history of seizures (recent or follow-up).
Exclusion criteria:
children outside the age group, i.e. < 5 years and > 16 years,
parents/guardians/children not consenting for the study.
The children were randomly assigned to MBIR and non-MBIR (based on FBP) groups.
Computed tomography paediatric head image acquisition
CT head examinations for the non-MBIR group were acquired on a Toshiba aquilionTM, 64-slice CT scanner, while for the MBIR group the images were acquired on a Philips Brilliance iCT 256-slice CT scanner using MBIR technology. The protocols for both the groups are summarized in Table 1. The images from both the study groups were transferred to a common workstation for analysis.
Table 1
Table summarizing the computed tomography protocols of non-model-based iterative reconstruction (MBIR) and MBIR groups
S. No. | Age group (in years) | Non-MBIR group | MBIR group | ||||||
---|---|---|---|---|---|---|---|---|---|
Mode | kVp | mA | Rotation time in seconds | Mode | kVp | mA | Rotation time in seconds | ||
1 | 5-10 | Axial | 120 | 180 | 1 | Axial | 120 | 200 | 0.5 |
2 | 10-16 | Axial | 120 | 260 | 1 | Axial | 120 | 300 | 0.5 |
Assessment of image-quality parameters
The scale for the assessment of image quality was based on a study done by Kilic et al. [15].
(i) Objective (quantitative) assessment:
Image noise was evaluated in air, cerebrospinal fluid (CSF), and white matter (WM). A 60 mm2 ROI was placed in the air (Figure 1A) within 1 cm of the scalp, at a place free from artefacts, and measurements were taken in 3 different regions (at the level of posterior fossa). Image noise was measured in CSF (Figure 1B) by keeping an ROI with an area of > 15 mm2 placed in lateral ventricles, or suitable CSF spaces if the measurement could not be performed in a lateral ventricle. The third ROI was measured in WM (Figure 1C) at the level of centrum semiovale placed in areas free of disease and distant from pathological sites.
Figure 1
Axial computed tomography images showing region of interest (ROI) placed in air (A), lateral ventricle (B), and grey matter (C)
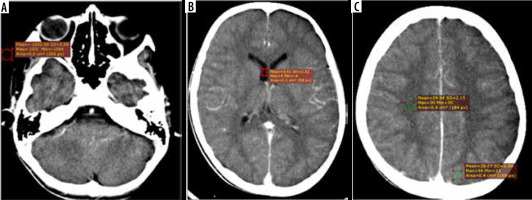
Contrast-to-noise ratio (CNR) was calculated by taking the means of 3 ROIs measured in grey matter (GM) (Figure 1C) and 3 in the WM measured at the level of centrum semiovale avoiding partial-volume effects. CTn and SD values were recorded and thereafter averaged for each part.
CNR = mean GM CTn – mean WM CTn/ [(mean SD in GM)2 + (mean SD in WM)2]1/2.
Signal-to-noise ratio (SNR) was calculated by first taking means of the CTn and SD in 3 ROIs in WM at the level of the centrum semiovale.
SNR WM = mean CTn/mean SD
ii) Subjective (qualitative) assessment:
Qualitative assessment was based on subjective image noise, image sharpness, diagnostic acceptability, and artefacts. The analysis was done by 2 radiologists independently (having 14 and 7 years of experience in paediatric radiology), after anonymizing non-MBIR and MBIR studies (blinded to all clinical, technical, and personal medical information). The images were analysed in a randomized order. A window level of 40-60 HU and window width of 60-90 HU was maintained for all the images. Each subjective parameter of image analysis was assessed on a scale of 4 to 5 points described as follows:
Noise: 1 – least, 2 – optimum, 3 – above average, 4 – too much noise, no information provided.
Sharpness: 1 – above average sharpness, 2 – average sharpness, 3 – below average sharpness, 4 – not sufficient for diagnosis, 5 – structures cannot be identified.
GM and WM differentiation, basal ganglia, pons, and margins of the sulci/gyri were used to assess the sharpness.
Diagnostic acceptability: 1 – fully acceptable, 2 – probably-acceptable, 3 – acceptable but under limited conditions, 4 – not acceptable.
Artefacts: 1 – none, 2 – minor, 3 – major but interpretable, 4 – image interpretation not possible.
Radiation dose estimates
The effective dose-mSv (ED) was calculated by multiplying the dose length product with an age-wise value of the conversion factor for CT head examinations according to ICRP Publication 60 Recommendations [16].
We used 0.0031 as a conversion factor for the age group 5-10 years and 0.0023 for 10-16 years in our study.
Statistical analysis
All the statistical analysis was done using Statistical Package for the Social Sciences (Version 22.0). An unpaired Student t-test was used for continuous variables. Wilcoxon rank sum (Mann-Whitney U) test was used for categorical variables. The kappa test and c2 test were applied to assess the subjective parameters determined by the 2 radiologists. All the statistical tests were 2-sided, performed at a significance level of α = 0.05.
Results
Age distribution
There were 41 children in the MBIR group, of whom 22 were 5-10 years old and 19 were 10-16 years old. There were 47 children in the MBIR group, of whom 33 were 5-10 years old and 14 were 10-16 years old. The difference in sample size in both groups was not statistically significant (p > 0.05).
Radiation dose analysis
The radiation exposure in terms of effective dose (ED), dose length product (DLP), and CT dose index volume (CTDIvol) showed a significant reduction in the MBIR group compared to the non-MBIR group.
There was an overall reduction in mean effective dose in the MBIR group by 81%. The reduction was similar in both the age groups (81.6% in 5-10 years, 80.3% in 10-16 years).
The overall reduction in mean DLP was 79.8%. The reduction in DLP was also similar in both the age groups (81.6% in 5-10 years, 80.3 % in 10-16 years).
The mean CT dose index volume was also reduced in the MBIR group, and the overall reduction was 88.5%. The age-wise reduction in CTDIvol in both the age groups was similar (89.6% in 5-10 years, 88.8% in 10-16 years).
All the reductions were statistically significant (p < 0.05).
Table 2 summarizes the mean ED, DLP, and CTDIvol in the MBIR and non-MBIR groups. Table 3 summarizes the age-wise comparison of radiation dose parameters between the MBIR and non-MBIR groups.
Table 2
Table summarizing the mean effective dose (ED), dose length product (DLP), and computed tomography dose index volume (CTDIvol) in the model-based iterative reconstruction (MBIR) and non-MBIR groups
MBIR group | Non-MBIR group | p-value | ||||
---|---|---|---|---|---|---|
Mean | SD | Mean | SD | |||
CTDIvol (mGy) | 9.85 | 2.02 | 85.86 | 17.00 | 0.0001 | |
DLP (mGy cm) | 132.59 | 31.03 | 656.86 | 145.12 | 0.0001 | |
ED (mSv) | 0.35 | 0.04 | 1.84 | 0.28 | 0.0001 |
Table 3
Age-wise comparison of radiation dose parameters between model-based iterative reconstruction (MBIR) and non-MBIR group
Objective image analysis
Table 4 summarizes objective image analysis in the 2 groups. No statistically significant difference was seen with regards to measured noise in WM and CNR. The overall SNR was higher in the non-MBIR group. The overall measured noise in air and CSF was lower in the MBIR group. The difference in measured noise in CSF in the 2 study groups in the 10-16-years age group was not statistically significant (p > 0.05).
Table 4
Table summarizing the mean measurement of noise (objective analysis)
Subjective image analysis
There was very good interobserver strength of agreement for the subjective image noise (K-0.833, p < 0.05, Table 5), subjective image sharpness (K-0.824, p < 0.05, Table 6), diagnostic acceptability (K-0.822, p < 0.05, Table 7), and for artefacts (K-0.802, p < 0.05, Table 8).
Table 5
Comparison of the subjective assessment of image noise done by Radiologist 1 and Radiologist 2
Table 6
Comparison of the subjective assessment for image sharpness done by Radiologist 1 and Radiologist 2
Table 7
Comparison of the subjective assessment of diagnostic acceptability done by Radiologist 1 and Radiologist 2
Table 8
Comparison of the subjective assessment for artefacts done by Radiologist 1 and Radiologist 2
The subjective assessments of image quality by the 2 ra-diologists were compared and are summarized in Figure 2.The differences between inter-observer ratings were not statistically different between the MBIR and non-MBIR groups except for the subjective image noise assessment by both the radiologists (p = 0.040). None of the MBIR/non-MBIR was rated as 4 or 5 for any of the parameters.
Discussion
In the present study, the potential of MBIR to reduce radiation-related dose parameters (ED, DLP, and CTDIvol) compared to conventional filtered back projections was assessed, and we found an overall reduction of 81% in mean ED, 79.8% in DLP, and 88.5% in CTDIvol. There was overall 81% reduction in mean ED, 79.8% in mean DLP, and 89% in mean CTDIvol in the MBIR group in our study. This is consistent with studies performed previously in adult body imaging [16]. No significant differences in the reduction in radiation dose parameters were seen in the 2 different age groups in the present study. No statistically significant difference was found in the image quality between the 2 groups in our study. Although the SNR was higher in the non-MBIR group, the overall noise in air and CSF was lower in the MBIR group; these findings are not significant because there was no effect on diagnostic acceptability. In addition, subjective assessment of image quality was similar in both the study groups and had a high inter-observer agreement. Lower measured noise in MBIR has been documented and published in previous studies [16], but no significant differences were documented in the results of the majority of subjectively assessed image quality parameters and in diagnostic acceptability.
Multiple techniques have been incorporated in an attempt to reduce the radiation dose while performing head CTs, which include but are not limited to reducing mAs, shields, angling the gantry to exclude the orbits, and automatic tube current modulation. Reducing mAs to reduce radiation dose goes hand in hand with increasing noise, thus leading to reduced image quality and interpretability. But if we reduce the noise by creating specific adaptive filters, we get an opportunity to reduce the mA and hence the radiation dose. MBIR reduces the radiation dose and at the same time maintains the image quality. There have been encouraging results with MBIR in chest and body imaging in the adult population [17]. However, it has limited validation in the paediatric population. We have evaluated and tried to validate the role of MBIR in the paediatric population in the present study.
According to the American College of Rheumatology (ACR) paediatric CT accreditation standards, the maximum acceptable phantom CTDIvol dose for a paediatric head CT examination is 40 mGy (for a 1-year-old child). Furthermore, for paediatric head CT examinations the estimated mean reported CTDIvol values have been reported to be 27.3 mGy (95% CI, 24.4-30.1 mGy), with a DLP of 390.9 mGy cm [18]. The overall mean CTDIvol and DLP in our study were 9.85 mGy and 132.59 mGy cm, respectively. Hence, a significant reduction can be made in radiation doses by employing MBIR.
In a study done by Meivelle et al. [19], children with cystic fibrosis were studied with standard CT and moderately reduced-dose CT plus a minimum-dose CT. Standard CT images were reconstructed with the FBP technique, while low-dose CT images were reconstructed with both FBP and VEO (MBIR). The images were subjected to qualitative assessment by radiologists as well as objective assessment. Compared to FBP images, VEO images showed significantly lower SD (p < 0.001) and higher SNR (p < 0.05). Our study also showed significant reduction in image noise (p < 0.5), whereas the SNR was lower in the MBIR group.
The reduction in radiation doses in MBIR is better compared to adaptive statistical iterative reconstruction (ASIR). Vorona et al. [8] calculated a 22% reduction in average CTDIvol and a 24% reduction in average DLP in paediatric head CT, while Kilic et al. [15] calculated a 30% reduction in average DLP in head CT using ASIR. Olcot et al. [10] found that in MBIR, perceived image quality was superior to ASIR and FBP overall (p < 0.001). MBIR achieved diagnostic overall perceived image quality with approximately half the radiation dose required by ASIR and FBP. The noise curve of MBIR was significantly lower and flatter (p < 0.001). They concluded that MBIR provides superior image quality at half the radiation dose. In our study also, none of the images was found be diagnostically unacceptable on subjective image analysis.
In the study by Katsura et al. [17], 100 adult patients underwent reference-dose and low-dose unenhanced CT scan of the chest. 50% ASIR-filtered back projection blending (ASIR50) was used for reconstruction of images for reference-dose CT, while both ASIR50 and MBIR were used for reconstruction of images for low-dose CT. The images were evaluated by the 2 radiologists for subjective image noise, artifacts, and diagnostic acceptability. Assessment of objective image noise was done in the lung parenchyma. A 79.0% decrease in dose-length product was seen with low-dose CT when compared to reference-dose CT. This study has determined the possibility of reducing the radiation dose with MBIR compared to ASIR and has again found a significant reduction in the dose.
The present study has some limitations. The scans were done on 2 CT scanners from different manufacturers, which may bias the findings. We did not include specific phantom data to assess radiation dose reduction. Theoretically, the possibility of comparing data from the same individual generated at 2 different points of time (for example, during follow-ups) exists, but this was not our primary objective and not practical due to time constraints. Also, we have measured noise in air, CSF, WM, and GM only. Noise measurements at other sites and at multiple levels could have been obtained to increase the degree of confidence; however, its practical utility and overall statistical difference remain unsubstantiated.